Scientists from an English laboratory have broken the record for the amount of energy produced during a controlled, sustained fusion reaction. The creation of 59 megajoules of energy in five seconds at the Joint European Torus (JET) experiment in England has been dubbed a “breakthrough” by certain news sites and has sparked considerable interest among physicists. However, a prevalent misconception about fusion electricity production is that it is “always 20 years away.”
We are nuclear physicists and nuclear engineers researching how to establish controlled nuclear fusion for the generation of power.
The JET result indicates great advances in our understanding of fusion physics. But, more crucially, it demonstrates that the novel materials employed to build the inner walls of the fusion reactor functioned as expected. The fact that the new wall construction performed so well distinguishes these results from past milestones and moves magnetic fusion from a pipe dream to a reality.
Particles joined together
Nuclear fusion is the combination of two atomic nuclei to form a single composite nucleus. This nucleus then disintegrates, releasing energy in the form of new atoms and particles that flee the reaction. The escaping particles would be captured and used to generate electricity by a fusion power plant.
On Earth, there are several methods for securely controlling fusion. Our research focuses on JET’s technique, which involves utilizing enormous magnetic fields to confine atoms until they reach a high enough temperature to fuse.
The fuel for existing and future reactors is two different isotopes of hydrogen, deuterium and tritium, which have the same proton but differing quantities of neutrons. The nucleus of normal hydrogen contains one proton and no neutrons. Tritium has one proton and two neutrons, whereas deuterium has one proton and two neutrons.
To have a good fusion process, the fuel atoms must first become so hot that the electrons break away from the nuclei. This results in plasma, which is a collection of positive ions and electrons. The plasma must next be heated until it reaches a temperature of more than 200 million degrees Fahrenheit (100 million Celsius). This plasma must then be held in a confined environment at high densities for an extended length of time in order for the fuel atoms to collide and fuse together.
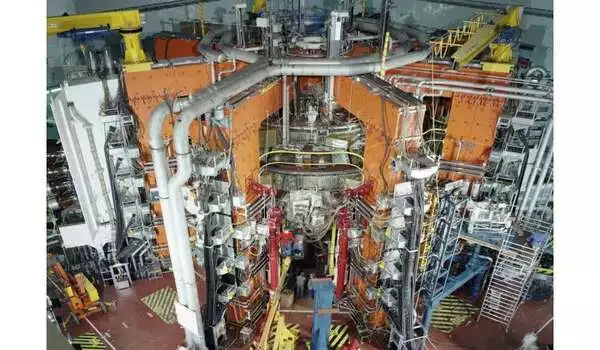
To manage fusion on Earth, researchers created tokamaks, which are donut-shaped devices that use magnetic fields to contain plasma. Magnetic field lines that wrap around the inside of the doughnut operate as train tracks that the ions and electrons follow. It is conceivable to accelerate the fuel particles to such high speeds that when they meet, instead of bouncing off each other, the fuel nuclei fuse together by infusing energy into the plasma and heating it up. This causes them to emit energy, primarily in the form of fast-moving neutrons.
Fuel particles migrate away from the hot, dense core during the fusion process and finally crash into the inner wall of the fusion vessel. To prevent the walls from deteriorating as a result of these collisions, which also pollute the fusion fuel, reactors are designed to guide the stray particles toward a heavily armored chamber known as the divertor. This eliminates any extra heat and pumps out the redirected particles, protecting the tokamak.
The walls are significant.
The fact that divertors can’t withstand the continual particle bombardment for more than a few seconds has been a fundamental constraint of previous reactors. To make fusion power commercially viable, engineers must construct a tokamak vessel that can withstand years of operation under fusion conditions.
The divertor wall is the first thing to think about. Despite the fact that the fuel particles are significantly cooler when they reach the divertor, they still have enough energy to knock atoms away from the divertor’s wall material when they collide with it. Previously, JET’s divertor had a graphite wall, but graphite absorbs and holds far too much fuel for practical use.
JET engineers upgraded the divertor and inner vessel walls to tungsten in 2011. Tungsten was chosen in part because it has the highest melting point of any metal, which is critical given that the divertor will be subjected to heat loads almost ten times greater than the nose cone of a space shuttle reentering the Earth’s atmosphere. The tokamak’s inner vessel wall was updated from graphite to beryllium. Beryllium offers good thermal and mechanical qualities for use in fusion reactors, absorbing less fuel than graphite while still withstanding high temperatures.
The energy produced by JET grabbed the headlines, but we’d argue that it’s the experiment’s use of new wall materials that makes it genuinely noteworthy, because future devices will require these more robust walls to function at high power for much longer periods of time. JET is a successful proof-of-concept for the next generation of fusion reactors.
The next generation of fusion reactors
The JET tokamak is the world’s largest and most advanced magnetic fusion reactor. The next generation of reactors, however, is already in the works, most notably the ITER experiment, which is scheduled to begin operations in 2027. ITER, which means “the Way” in Latin, is being built in France and is being sponsored and supervised by a multinational group that includes the United States.
Many of the material improvements demonstrated by JET will be used in ITER. However, there are some significant variances. First and foremost, ITER is huge. The fusion chamber is 37 feet (11.4 meters) tall and 63 feet (19.4 meters) in diameter, making it more than eight times the size of JET. Furthermore, ITER will use superconducting magnets, which can generate greater magnetic fields for longer periods of time than JET’s magnets. With these modifications, ITER is expected to break JET’s fusion records, both in terms of energy production and reaction duration.
ITER is also expected to do something fundamental to the concept of a fusion powerplant: generate more energy than is required to heat the fuel. Models suggest that ITER will generate 500 megawatts of power continuously for 400 seconds while needing only 50 MW of energy to heat the fuel. This meant that the reactor produced ten times more energy than it consumed, which was a significant improvement over JET, which took around three times the energy to heat the fuel than it produced for its recent 59 megajoule record.
Years of research in plasma physics and materials science have paid off, bringing scientists to the brink of harnessing fusion for power generation, as seen by JET’s recent track record. ITER will be a huge step toward the objective of industrial-scale fusion power plants.